
THE ROLE OF RNA METABOLISM
IN NEUROLOGICAL DISEASES Alaqeel AM1,2,*, Abou Al-Shaar H3, Shariff RK3, Albakr A2 *Corresponding Author: Ahmed M. Alaqeel, M.D., Department of Neurosurgery, University of Calgary,
1403 29th Street NW, Calgary, Alberta T2N 2T9, Canada. Tel: +403-970-9117. Fax: +403-270-7878. E-mail:
ahmedalaqeel@hotmail.com page: 5
|
INTRODUCTION
Neurodegenerative diseases are one of the leading
health challenges in modern medical history because
of their intriguing nature and the lack of knowledge
in the understanding of the underlying causes.
Recent research has helped scientists acquire a greater
insight into the cellular, molecular and genetic predispositions
of these conditions. The most difficult
aspect in treating these diseases is the time required
to make a proper diagnosis, which is usually when the
disease has already advanced to a stage of irreparable
damage. The studies carried out in the last 15 years
have helped scientists gain a better insight into the
molecular and genetic levels of predisposing factors
and disease mechanisms. Once the actual cause and
the pattern of disease progression are discovered,
the treatment options will become more available
and effective.
RNA metabolism is the basic process by which
RNA is generated, transported, regulated, stored and
translated. Recent studies have shown that mutations
in RNA-binding proteins are a key cause of several
human neuronal based diseases.
The major contributing factors to these neurodegenerative
diseases include pathogenic mutations,
amyloid precursor proteins, superoxide dismutase,
and DNA and RNA binding proteins. Additionally,
the most prominent factor associated with these
diseases is age. Studies have linked several genetic
risk factors with several neurological diseases, which
predispose individuals to develop these conditions
[1,2]. Table 1 depicts the role of RNA in various neurological
diseases. The literature states that there are
some common molecular events that happen during
the development and progression of these neurodegenerative
disorders, whether similar or different.
One of the common molecular events is oxidative
stress, which affects protein folding, aggregation and
degradation of some protein species, lipid peroxidation,
calcium homeostasis and DNA repair. Molecular
events also include protein oligomerization and aggregation,
axonal transport deficits, mitochondrial
dysfunction, excitotoxicity, calcium dysregulation,
neuron-glial interactions, neuroinflammation, DNA
damage and aberrant RNA processing.
RNA Translation in Neurodegeneration. Some
researchers have proposed that the synaptic efficacy
of neuronal dendrites and dendritic spines may depend
on local (synaptodendritic) protein synthesis
due to the presence of mRNA and polyribosomes [3].
In situ hybridization studies have further strengthened
the concept of local translation by demonstrating
the selective localization of some mRNA transcripts
to dendrites. These studies now suggest that certain
RNA forms are packaged into discrete ribonucleoproteins
(RNPs) that are then transported to the dendrites
where they are selectively expressed [4].
Synapses and synaptic networks are affected by
local translational control. This is demonstrated in
different studies wherein synapse-stimulating agents
have been found to elicit protein synthesis [5], while
protein synthesis inhibitors disrupt synaptic plasticity
including long-term potentiate (LTP), long-term
depression (LTD), and long-term facilitation [6].
Long-lasting late-phase LTP (L-LTP) is a form of
LTP that requires both gene transcription and RNA
translation [7].
The three major factors that control translation
in neurons, namely miRNAs, fragile X mental retardation
(FMRP), and cytoplasmic polyadenylation
[poly(A)] element-binding (CPEB) proteins are now
being investigated in detail to understand their mechanisms
and interactions [8]. Cytoplasmic poly(A)
element-binding is a sequence-specific RNA-binding
protein that represses translation until stimulated.
Once stimulated by signaling events, it leads to translation
activation by elongation of poly(A) tails of
mRNA [9]. Another associated factor is cytoplasmic poly(A) complex, which in turn affects poly(A)-induced
translation of mRNAs in dendrites in response
to synaptic stimulation [10]. The importance of these
cytoplasmic poly(A) complexes in synaptic function
can be observed by the changes in synaptic plasticity,
which occur in response to depletion of poly(A)
complex factors [9]. The CPEB displays a coherent
posttranscriptional molecular mechanism that underlies
essential brain functions.
RNA-Mediated Toxicity. Unstable trinucleotide
repeat expansions in the associated genes have been
found to be the cause of a large number of neurodegenerative
diseases [11]. These disorders can be classified
into different groups based on the pathogenic
mechanisms such as RNA toxicity, loss of protein
function and dominant protein-based toxicity.
RNA toxicity has been found to be responsible
for two neurodegenerative diseases named myotonic
dystrophy type 1 (DM1) and myotonic dystrophy
type 2 (DM2). The CUG and CCUG RNA expansions
in the non coding regions of the dystrophia
myotonica-protein kinase (DMPK) gene and the zinc
finger 9 gene (ZNF9), result in DM1 and DM2, respectively
[12].
Polyglutamine (polyQ) diseases such as Huntington’s
disease (HD) and the spinocerebellar
ataxias (SCAs) (such as SCA1, 2, 3, 6, 7 and 17)
are inherited disorders caused by expanded CAG
repeats within the coding region of the associated
genes [13]. A prominent pathological trait of these
diseases is the ubiquitinated nuclear inclusions (NIs)
containing chaperones and subunits of the proteasome
[13]. Research has predominantly focused on
protein-based mechanisms of toxicity caused by the
expanded polyQ domain. Recent studies are now suggesting
that RNA toxicity is the principal reason for
degeneration [14]. In one study, it was observed that
the toxicity of a pathogenic expanded polyQ Ataxin-3
protein was significantly contained by interrupting
the typically pure CAG-repeat sequence encoding
the polyQ domain with a CAA-interrupted repeat that
also encodes for glutamine. It has been proposed that
the toxicity is caused by the hairpin structure formed
by the CAG-repeat of RNA [15]. Interrupting the
CAG-repeat RNA by CAA codons has been indicated
to disrupt the hairpin structure [16]. Some old studies
have shown that pure or interrupted CAG-repeat
expansions in ATAXIN-2 (ATXN2) are linked with
distinct effects [17]. Expanded pure CAG repeat in
ATXN2 typifies SCA2. CAA interruptions in ATXN2
have been found in CAG-repeat expansions that are
associated with amyotrophic lateral sclerosis (ALS)
and Parkinsonism. These findings clearly indicate the
significance of the CAG-repeat RNA in toxicity and
its effects. Studies also revealed that a non coding
RNA bearing only an abnormally long CAG repeat,
but not coding for a protein, can result in progressive
neuronal dysfunction including shortened life span
and locomotor defects [11].
A set of studies with drosophila have shown that
muscleblind (mbl), an RNA-binding protein (implicated
as a modifier in DM1), can enhance the toxicity
of both Ataxin-3 protein and non coding CAG-repeat
RNA [18]. These observations clearly indicate that
the CAG-repeat RNA, in the absence of coding for
a protein, can become a source of toxicity in polyQ
diseases.
Roles of MicroRNAs in Neurodegeneration. It
is now an established fact that microRNAs (miRNAs)
have a significant role in neurodegeneration [19,20].
Micro-RNAs are the small endogenous RNAs that
act as post-transcriptional regulators of gene expression.
MicroRNAs primarily serve as guide molecules
for miRNA-containing ribonucleoprotein (miRNP)
complexes to reach their target mRNA. The binding
of the miRNP to the target in turn affects the expression
of the target mRNA [21]. The target levels of
expression are reduced either by the inhibition of the
translation of the target mRNA or by the destabilization
caused by mRNA deadenylation [22]. There
have been observations of up-regulation of targets
by miRNA [23].
Studies indicate that miRNAs have a huge regulatory
potential as they target hundreds of proteincoding
genes [24,25]. Many miRNAs are expressed
in the central nervous system in a temporally- and/
or spatially-regulated manner during development
[26]. Research has estimated that >1000 miRNAs
are expressed in the human brain [26].
Recent years have seen a rise in research on
miRNAs and their role in normal development, differentiation,
functions, as well as in pathology. MicroRNA
target sites are now being recognized for
single nucleotide polymorphisms (SNPs) associated
with various neural diseases [27]. MicroRNAs provide
the sequence information that guides the miRNP
to target mRNAs. Base-pairing interactions involving
six to eight residues at the 5’ end of the miRNA, called the “seed”, are thought to provide most of the
target recognition specificity with additional basepairings
contributing to the magnitude of regulation
[25]. Target sites are mainly observed in the non coding
portion of mRNAs [in 3’ untranslated regions
(3’UTRs)]. Change in one residue in a 3’UTR result
in a new seed match that leads to reduced expression
of that allelic form of the mRNA. Similarly, a loss
of a miRNA site could be caused by a 3’UTR SNP,
which in turn will result in an elevated expression of
that allele. A study identified an SNP that creates a site
for human miR-659 in the 3’UTR of the progranulin
gene, as a risk factor in a specific form of dementia
[28]. Another study reported an SNP that destroys a
site for miR-433 in the 3’UTR of FGF20 and has been
linked to an increased risk of Alzheimer’s disease
(AD) caused by overexpression of a-synuclein [29].
Research studies are now progressively implicating
miRNAs in biological processes linked to neurodegenerative
diseases. An occurrence of the polyQ
repeats in many proteins has been found to be directly
linked to neurode-generation [30]. Bilen et al. [31]
reported that depletion of dicer in HeLa cells significantly
enhanced pathogenic Ataxin-3-induced toxicity.
The adverse effects could be partially reduced
by complementing the cells with the purified small
RNA fraction containing total HeLa cell miRNAs
[31]. The polyQ disorder SCA1 results in the death
of cerebellar Purkinje cells [30]. In a research study,
depletion of dicer from mouse Purkinje neurons did
not impair cell survival in young mice, but when
they aged beyond 13 weeks, Purkinje neurons began
to die with degeneration and apoptosis. These mice
displayed mild ataxia that aggravated with age [32].
MicroRNAs have also been associated with
loss of dopaminergic neurons in Parkinson’s disease
(PD). MiR-133b was found to be expressed at
reduced levels in brains and in animal models with
PD [33]. Overexpression of miR-133 inhibited the
differentiation of embryonic stem cells into dopaminergic
neurons, while its depletion, using antisense
oligonucleotides, increased the expression of dopaminergic
neuronal markers. The transcription factor
pitx3 works as a target for miR-133b, confirming that
pitx3 thus regulates miR-133b expression, providing
a feedback loop, which is essential for normal control
of gene expression in this important cell type.
Whether misregulation of miR-133b is linked to clinical
disease remains to be determined.
Patients with dentatorubral-pallidoluysian atrophy
(DRPLA) have expanded polyQ repeats of
human Atrophin-1, which are the reason for neuronal
apoptosis. In drosophila studies, it was found that
miR-8 sets the level of Atrophin expression by binding
to its 3’UTR. Experimental drosophila lacking
miR-8 performed poorly in an assay for motor coordination
and their performance gradually declined with
age. There were abnormal cell deaths observed in the
brains of these flies. These defects were amended by
restricting the degree to which Atrophin is overexpressed,
which in turn indicates that elevated Atrophin
levels are responsible for the neurodegenerative
defects [34]. The miR-8 in drosophila is related to
human miR-200b and miR-429, which are known to
target the Atrophin orthologue arginine-glutamic acid
dipeptide repeats encoded (RERE) [34]. This RERE
has been demonstrated to bind to Atrophin-1 resulting
in its overexpression and subsequent apoptosis
in neuronal cell cultures [35].
Repeat Expansion Diseases and RNA Metabolism.
The expansion of the polymorphic repeat sequences
has been found to be the causative agent for
over 20 human diseases. It beholds several types of
SCA, HD and myotonic dystrophy [36]. The patients
inherit a repeat number that exceeds the pathogenic
threshold. The age-of-onset and the severity of diseases
are associated with the repeat length expansion
that happens within successive generations [36]. The
expansion is caused by the loss of repeat-containing
gene function, and recessive inheritance, and by the
dominant repeat-mediated mechanisms [37]. Many
observations signify mutational causes in multiple
related neurodegenerative diseases. These include
similar genetic and clinical features [30,36].
In many of the neurodegenerative diseases, the
expanded CAG trinucleotide repeats are identified
within a coding region. This results in an expanded
polyQ tract in the final protein [36]. Polyglutamine
can cause abnormalities due to the gain-of-function
interactions inherent to the expanded protein tract and
alterations to normal protein function [38]. However,
in some disorders, repeats do not encode polyQ, share
similar repeat sequences, pathogenic thresholds, or
clinical features with the ‘polyQ’ diseases. In these
diseases, the gain-of-function properties of the expanded
repeat RNA transcript is the main source for
the pathology, moreover, it consists of diseases such as
Huntington’s disease-like-2 (HDL-2), DM1 and DM2,SCA 8, 10, 12, and fragile X tremor/ataxia syndrome
(FXTAS) [11,39]. Some animal studies demonstrate
how the repeat RNA is able to cause pathology in the
animals as well as cellular model systems, through
multiple and possibly distinct pathways [14,17].
Pathology caused by expanded repeat RNA was
first reported in DM1. These pathologies were caused
by a non coding CUG repeat within the 3’UTR of the
DMPK gene. In DM2, it was found to be caused by a
non coding CCUG repeat within an intron of ZNF9
(CNBP) [40]. These expanded repeat RNA transcripts
form a ‘hairpin’ secondary structure that binds and sequesters
specific RNA-binding proteins [18]. The most
extensive characteristic of these is mbl-like 1 (MBNL-
1), which is involved in the regulation of alternative
splicing [41]. Sequestration of MBNL-1 results in the
missplicing of certain transcripts and subsequently
resulting in disease [12]. The pathology is associated
with the formation of nuclear RNA foci that colocalize
with MBNL-1 and are the sites at which sequestration
occurs [18,42]. Some other proteins have also been
identified that are bound or misregulated by repeat
RNA, including Pur alpha [43], CUG-binding protein
(CUG-BP) [44], and some heterogeneous nuclear ribonucleoproteins
(hn RNPs) [45].
The colocalization of MBNL-1 with RNA foci
and the dysregulation of alternative splicing have
been observed in human tissues and animal models
of the untranslated repeat diseases such as SCA10
[45], DM1, DM2 [12], SCA8 [46], FXTAS [47], and
HDL-2 [48]. The sequestration of MBNL-1 and dysregulation
of splicing may be a contributing factor to
many disorders. Moreover, RNA foci that colocalizes
with MBNL-1 is observed in human HD cells
[48] It further emphasizes the supporting role for this
pathway in the polyQ diseases.
Studies have identified the RNA repeats distinct
from RNA-binding protein sequestration that can result
in different disorders. Some studies have shown
the pathogenic role of bi-directional transcription;
it produces complementary repeat transcripts that
hybridize to form double-stranded RNA [17]. Modification
in Dicer-2 levels has been found to affect the
pathology and is associated with the formation of
21 nucleotide repeat RNAs, highlighting a role for
small RNA processing pathways. Bi-directional transcription
is involved in a number of diseases and this
pathway may be a common contributor to a dominant
pathology [49].
Another alternative mechanism that may be
responsible for some pathologies is the non ATG
initiated translation in all three reading frames [50].
This process combined with bi-directional repeat
transcription may be a contributing factor in many
pathologies through the production of multiple homopolymeric
proteins [50]. As the expanded repeat
disease involves late onset of neurodegeneration and
loss of affected tissue, access to samples and the detection
of early changes in pathology becomes difficult.
Therefore, human studies are limited and animal
models became an essential in investigating pathology.
Drosophila is a good model for expanded repeat
disease and shares a number of key pathways that are
similar to that of humans [14,17,38,51]. The studies
with these models have shown that repeat RNA
transcripts may contribute to dominantly inherited
human pathology through multiple pathways [17].
Frontotemporal dementia (FTD) and ALS have
similar genetic, clinical, and pathologic features [52].
The majority of patients with FTD develop features
of motor neuron dysfunction [52], and nearly half of
those with ALS are found to have evidence of frontal
lobe impairment [52]. Amyotrophic lateral sclerosis
is a fatal degenerative illness primarily affecting motor
neurons, while FTD is a progressive dementing
illness affecting neurons of the frontal, insular, and
anterior temporal cortex [36]. Some individuals with
FTD may incline to develop ALS [53]. The common
factor in the pathogenesis of the two diseases is
found to be trans-activation response (TAR) element
DNA-binding protein 43 (TDP-43) [54]. Both ALS
and FTD are characterized by abnormal accumulations
of TDP-43 [37]. The most common pathological
subtype of FTD is referred to as frontotemporal lobar
degeneration with TDP-43 (FTLD-TDP) [55].
A number of families have been reported with
an autosomal dominant pattern of disease in which
affected members may develop either FTD, ALS,
or both (FTD-ALS). Many of these families show a
genetic linkage to a region on chromosome 9p21 [56].
This chromosomal region is identified with both ALS
and FTD in many research studies. This illustrates the
genetic defect at chromosome 9p may be responsible
for both FTD and ALS [57,58].
The expansion of GGGGCC repeats is the most
common genetic cause of ALS and FTD with a prevalence
of approximately 5.0-7.0% in European and
North American cohorts [59]. It accounts for approximately 40.0% of familial ALS and 20.0% of familial
FTD. The age-related progression of ALS and FTD
along with the noncoding nature of the GGGGCC
expansion is very much similar to other noncoding
nucleotide repeat disorders, such as myotonic dystrophy,
FXTAS, and SCAs 8, 10 and 12.
|
|
|
|

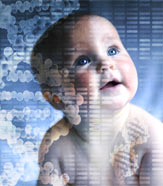

 |
Number 27 VOL. 27 (2), 2024 |
Number 27 VOL. 27 (1), 2024 |
Number 26 Number 26 VOL. 26(2), 2023 All in one |
Number 26 VOL. 26(2), 2023 |
Number 26 VOL. 26, 2023 Supplement |
Number 26 VOL. 26(1), 2023 |
Number 25 VOL. 25(2), 2022 |
Number 25 VOL. 25 (1), 2022 |
Number 24 VOL. 24(2), 2021 |
Number 24 VOL. 24(1), 2021 |
Number 23 VOL. 23(2), 2020 |
Number 22 VOL. 22(2), 2019 |
Number 22 VOL. 22(1), 2019 |
Number 22 VOL. 22, 2019 Supplement |
Number 21 VOL. 21(2), 2018 |
Number 21 VOL. 21 (1), 2018 |
Number 21 VOL. 21, 2018 Supplement |
Number 20 VOL. 20 (2), 2017 |
Number 20 VOL. 20 (1), 2017 |
Number 19 VOL. 19 (2), 2016 |
Number 19 VOL. 19 (1), 2016 |
Number 18 VOL. 18 (2), 2015 |
Number 18 VOL. 18 (1), 2015 |
Number 17 VOL. 17 (2), 2014 |
Number 17 VOL. 17 (1), 2014 |
Number 16 VOL. 16 (2), 2013 |
Number 16 VOL. 16 (1), 2013 |
Number 15 VOL. 15 (2), 2012 |
Number 15 VOL. 15, 2012 Supplement |
Number 15 Vol. 15 (1), 2012 |
Number 14 14 - Vol. 14 (2), 2011 |
Number 14 The 9th Balkan Congress of Medical Genetics |
Number 14 14 - Vol. 14 (1), 2011 |
Number 13 Vol. 13 (2), 2010 |
Number 13 Vol.13 (1), 2010 |
Number 12 Vol.12 (2), 2009 |
Number 12 Vol.12 (1), 2009 |
Number 11 Vol.11 (2),2008 |
Number 11 Vol.11 (1),2008 |
Number 10 Vol.10 (2), 2007 |
Number 10 10 (1),2007 |
Number 9 1&2, 2006 |
Number 9 3&4, 2006 |
Number 8 1&2, 2005 |
Number 8 3&4, 2004 |
Number 7 1&2, 2004 |
Number 6 3&4, 2003 |
Number 6 1&2, 2003 |
Number 5 3&4, 2002 |
Number 5 1&2, 2002 |
Number 4 Vol.3 (4), 2000 |
Number 4 Vol.2 (4), 1999 |
Number 4 Vol.1 (4), 1998 |
Number 4 3&4, 2001 |
Number 4 1&2, 2001 |
Number 3 Vol.3 (3), 2000 |
Number 3 Vol.2 (3), 1999 |
Number 3 Vol.1 (3), 1998 |
Number 2 Vol.3(2), 2000 |
Number 2 Vol.1 (2), 1998 |
Number 2 Vol.2 (2), 1999 |
Number 1 Vol.3 (1), 2000 |
Number 1 Vol.2 (1), 1999 |
Number 1 Vol.1 (1), 1998 |
|
|